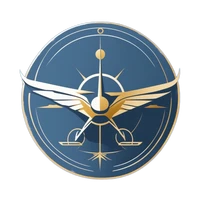
SkyXIntl/SkyReach_Companion_AI
Updated
•
2
text
stringlengths 3
32.8k
|
---|
ATPL GROUND TRAINING SERIES
POWERPLANT
Introduction
I
ii
I
Introduction
© CAE Oxford Aviation Academy (UK) Limited 2014
All Rights Reserved
This text book is to be used only for the purpose of private study by individuals and may not be reproduced in
any form or medium, copied, stored in a retrieval system, lent, hired, rented, transmitted or adapted in whole or
in part without the prior written consent of CAE Oxford Aviation Academy. Copyright in all documents and materials bound within these covers or attached hereto, excluding that material
which is reproduced by the kind permission of third parties and acknowledged as such, belongs exclusively to CAE
Oxford Aviation Academy. Certain copyright material is reproduced with the permission of the International Civil Aviation Organisation, the
United Kingdom Civil Aviation Authority and the European Aviation Safety Agency (EASA). This text book has been written and published as a reference work to assist students enrolled on an approved
EASA Air Transport Pilot Licence (ATPL) course to prepare themselves for the EASA ATPL theoretical knowledge
examinations. |
Nothing in the content of this book is to be interpreted as constituting instruction or advice
relating to practical flying. Whilst every effort has been made to ensure the accuracy of the information contained within this book, neither
CAE Oxford Aviation Academy nor the distributor gives any warranty as to its accuracy or otherwise. Students
preparing for the EASA ATPL (A) theoretical knowledge examinations should not regard this book as a substitute
for the EASA ATPL (A) theoretical knowledge training syllabus published in the current edition of ‘Part-FCL 1’ (the
Syllabus). The Syllabus constitutes the sole authoritative definition of the subject matter to be studied in an EASA
ATPL (A) theoretical knowledge training programme. |
No student should prepare for, or is currently entitled to enter
himself/herself for the EASA ATPL (A) theoretical knowledge examinations without first being enrolled in a training
school which has been granted approval by an EASA authorised national aviation authority to deliver EASA ATPL
(A) training. CAE Oxford Aviation Academy excludes all liability for any loss or damage incurred or suffered as a result of any
reliance on all or part of this book except for any liability for death or personal injury resulting from CAE Oxford
Aviation Academy’s negligence or any other liability which may not legally be excluded. Printed in Singapore by KHL Printing Co. Pte Ltd
Introduction I
iii
Introduction
I
Textbook Series
Book
Title
Subject
1
010 Air Law
2
020 Aircraft General Knowledge 1
Airframes & Systems
Fuselage, Wings & Stabilising Surfaces
Landing Gear
Flight Controls
Hydraulics
Air Systems & Air Conditioning
Anti-icing & De-icing
Fuel Systems
Emergency Equipment
3
020 Aircraft General Knowledge 2
Electrics – Electronics
Direct Current
Alternating Current
4
020 Aircraft General Knowledge 3
Powerplant
Piston Engines
Gas Turbines
5
020 Aircraft General Knowledge 4
Instrumentation
Flight Instruments
Warning & Recording
Automatic Flight Control
Power Plant & System Monitoring Instruments
6
030 Flight Performance & Planning 1
Mass & Balance
Performance
7
030 Flight Performance & Planning 2
Flight Planning & Monitoring
8
040 Human Performance & Limitations
9
050 Meteorology
10
060 Navigation 1
General Navigation
11
060 Navigation 2
Radio Navigation
12
070 Operational Procedures
13
080 Principles of Flight
14
090 Communications
VFR Communications
IFR Communications
Introduction
I
iv
I
Introduction
v
Introduction I
Introduction
I
Piston Engines
1. Piston Engines - Introduction . |
1
2. Piston Engines - General . .11
3. Piston Engines - Lubrication . |
.41
4. Piston Engines - Cooling . .55
5. Piston Engines - Ignition . |
.65
6. Piston Engines - Fuel . .75
7. Piston Engines - Mixture . |
.91
8. Piston Engines - Carburettors . 101
9. Piston Engines - Icing. |
115
10. Piston Engines - Fuel Injection . 123
11. Piston Engines - Performance and Power Augmentation . |
133
12. Piston Engines - Propellers . 161
Gas Turbines
13. Gas Turbines - Introduction. |
197
14. Gas Turbines - Air Inlets. 217
15. Gas Turbines - Compressors. |
225
16. Gas Turbines - Combustion Chambers . 245
17. Gas Turbines - The Turbine Assembly . |
263
18. Gas Turbines - The Exhaust System. 277
19. Gas Turbines - Lubrication . |
291
20. Gas Turbines - Thrust . 307
21. Gas Turbines - Reverse Thrust . |
325
22. Gas Turbines - Gearboxes and Accessory Drives. 333
23. Gas Turbines - Ignition Systems . |
341
24. Gas Turbines - Auxiliary Power Units and Engine Starting . 349
25. Gas Turbines - Fuels . |
365
26. Gas Turbines - Fuel Systems. 373
27. Gas Turbines - Bleed Air. |
389
28. Revision Questions . 401
29. Index. |
447
Contents
ATPL Book 4 Powerplant
Introduction
I
vi
I
Introduction
1
1
Chapter
Piston Engines - Introduction
Introduction. 3
Terminology . 4
Dynamics . 4
Bernoulli’s Theorem. |
5
A Venturi Tube . 6
Constant Mass Flow (The Continuity Equation). 6
The Gas Laws . 7
Charles’s Law . |
7
The Combined Gas Laws . 7
The Application of the Combined Gas Law . 7
Diesel Engines . 8
Terms and Formulae . |
9
Piston Engines - Introduction
1
2
1
Piston Engines - Introduction
1
3
Piston Engines - Introduction
1
Piston Engines - Introduction
Introduction
Man’s early attempt at powered flight was thwarted by the lack of a suitable engine to provide
the necessary power. The steam engine widely in use at the time was heavy and inefficient. Combustion took place outside of the engine and much of the heat energy produced was
wasted to the atmosphere. In 1862 Beau de Rochas developed an engine where the combustion process took place inside
the engine, but in 1876 it was Nikolaus Otto who first succeeded in producing a working engine
based on the principle. |
The principle of operation of the engine is accomplished by inducing a
mixture of air and fuel into a cylinder, which is then compressed by a piston. The mixture is ignited and the rapid rise in temperature causes the gas pressure in the cylinder
to rise and forces the piston down the cylinder. Linear movement of the piston is converted
into rotary motion by a connecting rod and crankshaft. The burnt gases are then exhausted to
atmosphere. |
The engine converts heat energy into mechanical energy. Internal Combustion Engines fall into three main categories, compression ignition engines
(Diesels), two-stroke and four-stroke spark ignition engines and Wankel rotary engines. These notes cover in detail the construction and operation of the four-stroke engine which is
commonly used in aviation, and generally referred to as the Piston Engine. Figure 1.1
Figure 1.1
Before we look at the operation and construction of the piston engine an understanding of
the following terms, definitions and theories will be required. |
Piston Engines - Introduction
1
4
1
Piston Engines - Introduction
Terminology
Force:
A Force is that which, when acting on a body which is free to move, causes it to move, or
conversely, that which stops, or changes the direction of a moving body. Force is produced when a mass is accelerated. Force = Mass × Acceleration (F = m × a) e.g. A
force moves the piston down the cylinder (Units: newtons or pounds force). |
Work:
The Work Done by a force is defined as the product of the Force and the Distance moved in
the direction of the applied force. (Units: joules or foot pounds) e.g. The piston is moved from
the top to the bottom of the cylinder by a force. Energy:
Energy is the capacity of a body to do work. |
Energy comes in many forms: Heat, Light, Chemical, Kinetic, Potential. (Units: joules)
The Law of Conservation of Energy states that: “Energy can be neither created nor destroyed;
only its form may be changed”. The chemical energy of the fuel is converted to heat energy
during combustion in the engine. The engine then converts this to mechanical energy. |
Power:
Power is the rate of doing work. Work Done per unit time. (Units joules/second = watts or foot
pound/minute = horsepower) Work is done as the piston moves in the cylinder. It is moved so
many times a minute, and so the power can be measured. |
The horsepower is a measurement
of power which is equal to 33 000 foot pounds a minute. Dynamics
Newton’s Laws of Motion deal with the properties of moving objects (or bodies). It is easy to
see a piston or crankshaft move, but air is also a body, and will obey Newton’s Laws. It should
be remembered that air is the working fluid within the engine. |
First Law. “A body will remain at rest or in uniform motion in a straight line unless acted on by an
external force”. To move a stationary object or to make a moving object change its direction a force must be
applied. The mixture of fuel and air for a piston engine does not want to flow into the cylinder,
a force must make it flow. |
The piston moving down the cylinder does not want to stop. This
opposition of a body to change its motion or state of rest is called Inertia. Newton’s 1st Law
has no units of measurement. It is a property a body possesses, when stationary or moving. |
Newton’s 1st Law is known as the Inertia Law. Second Law. “The acceleration of a body from a state of rest, or uniform motion in a straight line, is
proportional to the applied force and inversely proportional to the mass”. The energy released by the fuel during combustion increases the pressure energy of the air
in the cylinder, and work can be done. |
The force to move the piston can be controlled by
1
5
Piston Engines - Introduction
1
Piston Engines - Introduction
changing the pressure in the cylinder. The mass of the piston is accelerated to a velocity. Mass
× Velocity is defined as Momentum. It is similar to inertia but only applies to moving bodies,
and has units of measurement. |
kg and metres per second. Newton’s second Law is known as
the Momentum Law. Third Law. “For every action there is an equal and opposite reaction”. |
Many examples of the application Newton’s third Law can be observed. The recoil of a gun as
the bullet is forced from its barrel, the snaking of a hose as water is forced from its nozzle, and
the operation of the jet engine. Newton’s third Law is known as the Reaction Law. Thermodynamics:
Is the study of Heat/Pressure energy. |
(Or the behaviour of gases and vapours under variations
of temperature and pressure). First Law. “Heat and Mechanical energy are mutually convertible and the rate of exchange is constant
and can be measured”. (If two moving surfaces are rubbed together without lubrication, heat will be generated and can
be measured with a temperature gauge. |
This is Mechanical energy converted into Heat energy,
conversely, when fuel is burned in a piston engine, the Heat energy in the fuel is converted to
Mechanical energy by the action of pistons and crankshaft. This too can be measured.) Second Law. “Heat cannot be transferred from a region at a lower temperature to one at a higher
temperature without the expenditure of energy from an external source”. |
(Heat will naturally flow from a radiator to the colder atmosphere which surrounds it, but the
expenditure of energy is required to lower the temperature of a refrigerator to a level below
that of the surrounding atmosphere.) Bernoulli’s Theorem
Daniel Bernoulli, a Swiss scientist (1700-1782), discovered certain properties relating to fluids
in motion. These were expressed in the mathematical statement that the total energy in a
moving fluid or gas is made up of three forms of energy - the energy due to the height or
position (the potential energy), the energy due to pressure, and the energy due to movement
(the kinetic energy) - and that in the streamline flow of an ideal fluid the sum of all these is
constant. When considering the flow of air the potential energy can be assumed to be constant; the
statement can therefore be modified, for all practical aerodynamic purposes, by saying that
the kinetic energy plus the pressure energy of a smooth flow of air is always constant. |
Thus,
if the kinetic energy is increased, the pressure energy drops proportionately so as to keep the
total energy constant. Piston Engines - Introduction
1
6
1
Piston Engines - Introduction
A Venturi Tube
A practical application of Bernoulli’s theorem with which the pilot should be familiar is the
Venturi tube, sometimes called a convergent/divergent duct (Figure 1.2) The Venturi tube has
an inlet which narrows to a throat, and an outlet section, relatively longer, which increases in
diameter towards the rear. Constant Mass Flow (The Continuity Equation)
For a flow of air to remain streamlined the volume passing a given point in unit time (the mass
flow) must remain constant; if a Venturi tube is positioned in such an airstream then, for the air
to remain streamlined, the mass flow through the Venturi must remain constant. Mass Flow is
dependent on the Area × Density × Velocity and is a constant. |
This is known as the continuity
equation. To do this and still pass through the reduced cross-section of the throat the speed of flow
through the throat must be increased. In accordance with Bernoulli’s theorem this brings
about an accompanying pressure and temperature drop. The use of Venturi tubes have many
applications in aircraft systems. |
For example the pressure drop at the throat of the Venturi
forms the basic principle of operation of the carburettor (Chapter 8). Figure 1.2 A Venturi
1
7
Piston Engines - Introduction
1
Piston Engines - Introduction
The Gas Laws
Boyle’s Law states that: “In a gas held at a constant temperature, the volume is inversely
proportional to the pressure.” or:
P × V = K
where P is the absolute pressure of the gas, and V is the volume occupied when the pressure
is P.
Hence the product of the absolute pressure and volume of a given quantity of gas is constant
when the temperature does not change. Charles’s Law
Charles’s Law, or Gay-Lussac’s Law states that: “If any gas is held at a constant pressure, its
volume is directly proportional to the absolute temperature”. V
T = K
The Combined Gas Laws
The Combined Gas Law is a combination of Boyle’s law and Charles’s Law and represents the
relationship between Volume, Pressure and Temperature. |
This may be shown as:
P × V
T
= K, alternatively, where K is the gas constant P × V = K × T
or
P1 × V1
T1
=
P2 × V2
T2
The Application of the Combined Gas Law
The changes in pressure, volume and temperature within the engine cylinder as the piston
moves between the top and the bottom of its stroke are illustrated in Figure 1.3 overleaf. These movements are known as the four strokes of an internal combustion engine (where
combustion takes place in the engine cylinder, and not externally as in the case of a steam
engine) as explained in the Otto cycle text which follows, it will be seen that only one useful or
power stroke is available during the cycle which occupies two revolutions of the crankshaft. It
will be appreciated that although the piston moves up and down the cylinder (“strokes”) four
times, there are,in fact, theoretically, five events in the cycle. Piston Engines - Introduction
1
8
1
Piston Engines - Introduction
Figure 1.3
Diesel Engines
Historically credit for the design of ‘cold-fuel’ compression-ignition does not lie with Rudolf
Diesel. |
In 1891 Herbert Akroyd Stuart invented the ‘cold-fuel’ injection system similar in operation to
modern-day automotive and aero-engine applications pre-dating Diesel’s design. In 1892 Rudolf Diesel designed and patented a similar engine to Akroyd Stuart’s known as
the ‘hot-bulb’ system where the fuel was introduced to the engine utilizing a compressed-air
delivery which ‘pre-heated’ the fuel allowing an easier start to be achieved. Thereafter although strictly Akroyd Stuart’s design the compression-ignition engines became
known as ‘Diesels’. Cold-fuel compression-ignition engines were developed further because
they can run faster, weigh less and are simpler to maintain. |
Diesel engines for use in aircraft are by no means a new idea. Aero-diesels appeared during
the late 1920s. The mechanical parts of the diesel engine are similar to those of a conventional gasoline-driven
engine with the exception that diesels reciprocating parts are slightly heavier in order to cope
with higher compression-ratios within. Recent developments in materials technology, superchargers and design have brought the
diesel to comparable weights with conventional engines and indeed, with even better power/
weight ratios. |
These developments have given way to recent certified retro-fits being trialled in
the Warrior PA28 and the Cessna 172. 1
9
Piston Engines - Introduction
1
Piston Engines - Introduction
Terms and Formulae
Quantity
Symbol
Standard Units
Formula
1
Potential Difference
V
Volts, V
V = IR
2
Current
I
Amperes, A
I = V/R
3
Resistance
R
Ohms, Ω
R = V/I
4
Power
P
Watts. W
P = V × I or
P = I2R
5
Force
F
Newtons, N
Pounds force, lbf
F = ma
6
Mass
m
Kilograms, kg
Pounds, lb
F = ma
7
Density
ρ
kg/m3 or lb/ft3
ρ = m/V
8
Moment
M
Newton Metres
Pounds Feet
M = F × d
9
Velocity
v
metres/sec ft/sec
v = d/t
10
Acceleration
a
m/sec2 or ft/sec2
a = F/m
11
Pressure
P
Pascals, Pa (N/m2)
lb/in2
P = F/A
12
Area
A
m2 or in2
A = F/P
13
Volume
m3 or ft3
14
Frequency
Hertz, Hz
cycles/sec
15
Work Done
Joules, J or ft lb
Wd = F × d
16
Potential Energy
PE
Joules, J
PE = m × g × h
17
Kinetic Energy
Joules, J
KE =1/2mv2
18
Efficiency
Useful work output
Total energy input
Piston Engines - Introduction
1
10
1
Piston Engines - Introduction
11
2
Chapter
Piston Engines - General
Engine Layout . .13
The Theoretical Otto Cycle . |
.14
The Operation of the Theoretical Otto Cycle . .16
The Operation of the Practical Otto Cycle. .17
Power to Weight Ratio . .22
Specific Fuel Consumption (SFC) . |
.22
Engine Efficiencies . .22
Compression Ratio . .23
Engine Construction . .24
The Crankcase. |
.24
The Crankshaft (Cranked-shaft) . .24
Connecting Rods . .26
The Pistons . .26
Cylinder Barrel or Block. |
.27
The Cylinder Head . .28
Valve Operating Gear. .28
Valve Clearance . .28
The Sump . |
.29
The Carburettor. .30
The Accessory Housing or Wheelcase . .30
Questions . .32
Answers . |
.40
Piston Engines - General
2
12
2
Piston Engines - General
2
13
Piston Engines - General
2
Piston Engines - General
Engine Layout
The power of an engine can be increased by adding cylinders producing multi-cylinder engines. This is a more efficient way of increasing power than making a single cylinder larger, and also
has the benefit of making the engine run smoother. There are various types of engine design
with regard to cylinder arrangement. Figure 2.1 Engine Layouts
Figure 2.1 Engine Layouts
-
The cylinder arrangement selected for a particular engine will depend on the type of cooling
of the engine, the power required, and role of the aircraft. |
Early aircraft used In-line engines. These have their cylinders arranged in a straight line, one after the other, they can be liquid or
air-cooled. The air-cooled variants are limited to around six cylinders. Many in-line engines are
inverted, so that the crankshaft is at the top and pistons below. |
The propeller is driven from the
crankshaft and this arrangement gave greater ground clearance for the propeller. The V Engine arrangement was used for larger more powerful engines of eight to twelve
cylinders. These engines powered the fighter aircraft of World War 2. Liquid-cooled, the V
arrangement of cylinders could easily be streamlined into the fuselage so reducing drag. |
The
liquid cooling system however increased weight and complexity of the engine. Like the in-line
engine they could also be inverted. The Radial Engine gave a large frontal area to the aircraft, but was short in length. The pistons
are arranged radially around a single-throw crank. |
Although drag was increased the engines
were light, rigid and produced high power. Piston Engines - General
2
14
2
Piston Engines - General
Radial engines always have an odd number of cylinders. By placing further rows of cylinders
behind the first produced Double and Triple Bank radials. These engines, although very
powerful, had the disadvantages of being heavy and presenting a large frontal area as they
were air-cooled. |
Most modern light aircraft use four or six cylinder engines arranged in the Flat/Horizontally
Opposed configuration. This arrangement makes for a short rigid engine, which is easily
streamlined. The Theoretical Otto Cycle
In the introduction, the basic principle of operation of the piston engine was explained. The
following paragraphs will explain in detail changes to the piston, valves, ignition and state of
the gas throughout the operation. |
It was stated that the engine works on a four stroke cycle. A Stroke is defined as the linear distance that the piston moves in the cylinder. When the piston
is at the top of the stroke it is said to be at Top Dead Centre (TDC), and when at the bottom
of the stroke Bottom Dead Centre (BDC). The piston is connected to a crankshaft. |
and as the piston moves from TDC to BDC the crankshaft
rotates 180°. The complete cycle taking 720° (4 × 180) The Stroke is equal to Twice the Crank-
throw. Figure 2.2 an engine which has a bore equal to the stroke is known as over-square. Figure 2.2
Figure 2.2
The internal diameter of the cylinder is called the Bore. |
These terms are used to explain the
Otto cycle. Piston and valve positions are related to degrees of crankshaft movement, and
position in relation to TDC and BDC. The four strokes of the Otto cycle are shown in Figure 2.3. 2
15
Piston Engines - General
2
Piston Engines - General
Figure 2.3 The four strokes of the Otto Cycle
Piston Engines - General
2
16
2
Piston Engines - General
The Operation of the Theoretical Otto Cycle
The four strokes are:
a)
Induction
b)
Compression
c)
Power
d)
Exhaust
When the piston is at TDC at the end of the compression stroke an electrical spark is produced
at the spark plug, and ignites the fuel air mixture. |
It should be appreciated that this does not
result in an explosion of the mixture, but is a controlled burning. This event is called Combustion. The combustion process takes place with the piston at TDC. The volume in the cylinder at that
moment in time is constant. |
Combustion is said to take place at Constant Volume. In the theoretical Otto cycle there are Five Events:
a)
Induction
b)
Compression
c)
Combustion
d)
Power
e)
Exhaust
These events can be shown graphically by a valve timing diagram - Figure 2.4. The timing
diagram shows the relationship between the events, and degrees of crankshaft rotation. Each
arc between TDC and BDC represents 180° of crankshaft rotation. |
Figure 2.4 The theoretical timing diagram for the Otto Cycle
2
17
Piston Engines - General
2
Piston Engines - General
The Operation of the Practical Otto Cycle
In practice the theoretical cycle proved to be inefficient and it was necessary to modify the
times of valve openings and closings and ignition. A typical practical timing diagram is shown
in Figure 2.5 and the reasons for the modified timings are discussed below. Figure 2.5 A practical valve and ignition timing diagram
The Induction Stroke
Opening the inlet valve before TDC ensures that the valve is fully open early in the induction
stroke, there is then no time-lag between the piston moving down and the mixture flowing
into the cylinder as would otherwise occur due to the inertia of the mixture. The inflowing
mixture can thus keep up with the descending piston. |
The momentum of the mixture increases as the induction stroke proceeds, and towards the
end of the stroke, it is such that the gases will continue to flow into the cylinder even though
the piston has passed BDC and is moving upwards slightly. The closing of the inlet valve is
therefore delayed until after BDC when the gas pressure in the cylinder approximately equals
the gas pressure in the induction manifold. The Compression Stroke
As the piston moves upwards, the inlet valve closes and the gas is compressed. By squeezing the
gas into a smaller space the pressure that it will exert when burnt is proportionally increased. |
It should be noted that as the gas is compressed it becomes heated adiabatically, in the same
way that a bicycle pump warms up in action, as well as by conduction from its hot surroundings,
and the pressure consequently rises to a higher value than that to be expected from the
reduction in volume alone. Piston Engines - General
2
18
2
Piston Engines - General
Adiabatic
Adiabatic means without loss or gain of heat. With present technology it is not possible to
compress or expand a gas without gain or loss of heat. The Power Stroke
Before the piston reaches TDC on the compression stroke the gas is ignited by a spark, the
momentum of the moving parts carrying the piston past the TDC whilst the flame is spreading. |
As the flame spreads through the combustion chamber the intense heat raises the pressure
rapidly to a peak value which is reached when combustion is complete, this coincides with the
piston being at about 10° past TDC. If the exhaust valve is not opened until BDC the pressure of the gases remaining in the cylinder
would create a back pressure resisting the upward movement of the piston. As the piston
descends on the power stroke, the pressure falls rapidly and by 45° of crank angle after TDC. is approximately half its peak value, and by 90° of crank angle after TDC. |
most of the energy
in the gases has been converted into mechanical energy. If the exhaust valve is opened before
BDC the residual pressure will start the first stage of exhaust scavenging, so that by BDC there
will be no back pressure on the piston. This pressure scavenging does not produce a significant loss of mechanical energy because:
a)
There is only a short distance left for downward movement of the piston after the
exhaust valve is opened. b)
Relatively little pressure is still being exerted on the piston by the cooled expanded
gases. |
The Exhaust Stroke
Finally the piston moves upward forcing the remaining gases out of the cylinder. The exhaust
valve is left open after TDC to permit the gases to scavenge the cylinder as completely as
possible by their momentum. About the position of TDC. and BDC, the distance the piston moves is very small compared
to the large angular movement of the crankshaft. |
This is called the Ineffective Crank Angle -
Figure 2.6. As there is little change in the cylinder volume at these times, the weight of charge
into the cylinder and the exhaust of the burnt gases can be improved by opening the valves
early and closing them late. These changes to the valve timing are named Valve Lead, Valve
Lag and Valve Overlap (see Figure 2.5). Valve Lead
Is when the valve opens before the theoretical opening time. |
(Inlet valve opens before TDC,
exhaust valve opens before BDC). Valve Lag
Is when the valve remains open after the theoretical closing time. (Inlet valve remains opens
after BDC, exhaust valve remains open after TDC). Valve Overlap
Is a period when both valves are partially open together. |
During this period the action of the
exhaust gases flowing out of the cylinder tends to reduce the gas pressure in the cylinder below
the gas pressure in the induction manifold. The mixture commences to flow into the area of
low pressure and assists in displacing the remaining burnt gases and by doing so improves the
volumetric efficiency of the engine by inducing a greater weight of charge into the cylinder. 2
19
Piston Engines - General
2
Piston Engines - General
The valve timing for a particular engine is fixed, and does not vary with engine speed. Control of power in the piston engine is achieved by varying the quantity of air which enters
the cylinder; this in turn will vary the pressure rise during combustion. |
The pilot controls a valve,
the Throttle to vary the quantity of air. Figure 2.6 Ineffective crank angle
The variations in pressure within the cylinder during the four strokes can be measured and
indicated graphically by a device which produces an Indicator diagram. The device plots
pressure against volume, and the graph is also known as a PV diagram. This small attachment,
fitted to research and experimental engines, consists basically of a pressure transmitter fitted
into the combustion chamber of the engine, (in a similar manner to a sparking plug), activating
a moving pen which traces cylinder pressure variation against piston position, as shown in
Figure 2.7. |
Figure 2.7. A Typical Indicator Diagram
Figure 2.7 A typical indicator diagram
Piston Engines - General
2
20
2
Piston Engines - General
The indicator diagram is used to plot the maximum pressures obtained, this determines the
shape and the area enclosed by the graph. This area is representative of the work done on the
air and the power produced. Figure 2.9 shows the indicator diagram opened out so that the pressure areas under the curve
can be more easily compared and measured. |
The area within the power column represents work done on the piston during the power
stroke and the blue areas represent work done by the piston in compressing the charge and
exhausting the cylinder against back pressure. This results in an average reading of pressure
on the piston during the working cycle being available which is termed the Indicated Mean
Effective Pressure (IMEP). The pilot is not given a display in the cockpit of the IMEP but what can be displayed is manifold
pressure which is representative of cylinder pressure. This is displayed on the manifold pressure
gauge. |
Opening the throttle increases manifold pressure and closing the throttle will reduce
it. The Manifold Absolute Pressure gauge (MAP) is normally calibrated to read in inches of
mercury. Figure 2.8 Manifold absolute pressure gauge
2
21
Piston Engines - General
2
Piston Engines - General
Figure 2.9 An indicator diagram plotted against stroke for simpler calculation of pressure areas
Having found the pressure in the cylinder it is now possible by calculation using the known constants,
area of piston, (bore), distance moved (stroke), number of cylinders and time. To calculate the
INDICATED HORSEPOWER (IHP) of the engine concerned, use the formula:
P × L × A × N × E
33 000
IHP =
where:
P = Indicated Mean Effective Pressure (lb/in2)
L = Length of Stroke (ft)
A = Area of cylinder (in2)
N = The number of cylinders
E = Effective working strokes/min (rpm)
In the introduction, power was defined as the rate of doing work. |
Work is done when a force
is moved through a distance. A force acts on the piston - (lb) The piston moves through the
distance of the stroke - (ft) It does this so many times a minute. This multiplies out as ft-lb per
minute. The inventor of the steam engine James Watt calculated that the average horse could move 1lb
a distance of 33 000 ft in 1 minute - (550 ft/lb/second). |
This is why P L A N E is divided by the
constant of 33 000 and the unit of power referred to as horsepower. The SI unit of power is the watt, and 750 watts is approximately equal to 1 horsepower. IHP is only a theoretical value of power. In moving the piston and turning the crankshaft power
is used. |
This is called Friction Horsepower, (FHP), and must be deducted from the IHP. The
power then left to do useful work (for example driving a propeller) is called Brake Horsepower
(BHP). Piston Engines - General
2
22
2
Piston Engines - General
Power to Weight Ratio
Power to Weight Ratio (Specific Power Output) is a comparison of an engine’s power output
per unit weight (kW/kg or horsepower/lb) expressed as a ratio. For example: An engine weighing 1000 lb (450 kg) and producing 250 hp (190 kW) would
produce a power to weight ratio of 0.42 kW/kg, or 0.25 hp/lb. |
Specific Fuel Consumption (SFC)
The increase in energy given to the air comes from the heat released by burning the fuel. This
in turn produces power in the engine. The weight of fuel burnt, in lb, for the power produced
BHP in unit time (hours) is called the Specific Fuel Consumption. Engine designers strive to get as much power as possible from the engine, for the minimum
weight of fuel burnt. |
During operation a reduction in power for the same weight of fuel burnt,
is defined as an Increase in Specific Fuel Consumption, and a reduction in fuel burnt for the
same, or more power a Decrease in Specific Fuel Consumption. SFC is affected by engine design and pilot operation of the engine. Since the pilot has no
control over design, correct operation of the engine is essential if performance figures are to
be attained. Engine Efficiencies
The engine is a machine that converts heat energy into mechanical energy. |
Sadly there are
losses in this transfer; engine design will try to reduce these losses. As stated previously the IHP
developed in the engine is reduced by FHP, leaving BHP to do useful work. The term efficiency means simply a comparison of what is got out of a system, with what is put
in to the system. The efficiency of any mechanical device must be less than unity, it is usual to
express it as a ratio. |
Mechanical Efficiency
Efficiency = Output
Input
× 100% Thus the mechanical efficiency = BHP
IHP × 100%
A typical value of mechanical efficiency would be in the region of 80 - 85%. Thermal Efficiency
The efficiency at which the heat energy released by the combustion of the fuel is converted to
work done in the engine is known as the Thermal Efficiency. Thermal Efficiency =
heat converted into work
heat energy available within the fuel × 100%
Engine design and the use of correct fuels increase thermal efficiency. A good value for thermal
efficiency in an internal combustion engine would be 25 - 28%. |
As previously stated, air is the working fluid within the engine. Added to this is fuel, so it
is actually a mixture of air and fuel that enters the cylinders. The power of the engine is
determined by the maximum weight of mixture (charge) induced, and the subsequent rise in
pressure during combustion. Due to inertia and factors affecting the density of the mixture, it
is not possible to fill the cylinder completely during the induction stroke. |
2
23
Piston Engines - General
2
Piston Engines - General
Volumetric Efficiency
The ratio of the weight of mixture induced to that which would fill the cylinder under normal
temperatures and pressures is called Volumetric Efficiency. × 100%
weight of mixture actually induced
weight of mixture which could fill cylinder
Volumetric =
Efficiency
at normal temperatures
and pressures. The volumetric efficiency of the engine is indicative of how well the engine is breathing. This
is affected by design, i.e. |
valve lead, lag and overlap. It is also affected by variables such as,
exhaust back pressure, resistance to flow and the force pushing the mixture into the cylinder. If the force is the difference in pressure between atmospheric and the cylinder pressure during
induction, the engine is said to be Normally Aspirated. A normally aspirated engine will have a volumetric efficiency of between 75-85% maximum. |
One way to improve the volumetric efficiency and hence power, is to increase the force pushing
the mixture into the cylinder. This is called Supercharging and is covered later in these notes. Compression Ratio
The work done on the mixture by the piston during the compression stroke depends on the
weight of mixture induced and the pressure that it is raised to. The pressure rise will depend on
the reduction in volume. |
There are three volumes that need to be considered. They are defined
below and illustrated in Figure 2.10. Figure 2.9
Figure 2.10
Total Volume is the volume above the piston when the piston is at BDC. Swept Volume is the volume displaced by the piston during a single stroke. |
Swept volume = cross-sectional area of the cylinder × the stroke. Clearance Volume is the volume above the piston crown when the piston is at TDC, this forms
the combustion chamber. Total Volume = Swept Volume + Clearance Volume. The increase in
pressure is called the Compression Ratio of the engine. |
Piston Engines - General
2
24
2
Piston Engines - General
The Compression Ratio is the ratio of the total volume enclosed in the cylinder with piston at
BDC, to the volume at the end of the compression stroke with the piston at TDC. Compression Ratio =
Total Volume
Clearance Volume
EXAMPLE. If the swept volume is equal to 1300 cc, and the clearance volume is equal to 200
cc the compression ratio would be equal to:
Total Volume = Swept Volume + Clearance Volume
Total Volume = 1300
+
200
Compression Ratio =
Total Volume
Clearance Volume
Compression Ratio = 1500
200
Compression Ratio = 7.5 : 1
Note: An increase in compression ratio will result in better fuel utilization (hence greater
Thermal Efficiency) and a higher mean effective pressure provided the correct fuel is used. This, however, will be at the expense of higher loading on the moving parts due to an increased
working pressure. |
Engine Construction
The main components of the engine were stated in the introduction. The following is a more
detailed explanation of the mechanical components and their function. The Crankcase
The crankcase is usually made in
two halves to make installation
and removal of the crankshaft
easier, it houses the main bearings
for the crankshaft, supports the
cylinders and provides mounting
faces
and
spigots
for
the
attachment of the other main
engine casings. Generally made of light alloy, it
forms a sealed chamber for the
lubricating oil, and is provided
with the means of attaching the
engine to its mounting frame in
the aircraft see Figure 2.11. |
A vent to atmosphere is normally
provided in order that gas
pressure build-up in the crankcase
is avoided. Figure 2.10
Vent
Crankcase
Figure 2.11
2
25
Piston Engines - General
2
Piston Engines - General
Crankshaft (Cranked-shaft)
The crankshaft, illustrated in Figure 2.12, converts the reciprocating or linear motion of the
pistons into rotary motion, and transmits torque to the propeller, and provides the drive for
accessories. The offset Crank Throw also determines the piston stroke. Figure 2.12 A four cylinder crankshaft
The Journals, the main part of the shaft, are supported by the main bearings in the crankcase. |
The Pistons are attached by the Connecting Rods to the Crank-pin. The crankshaft often has as many crank throws as there are pistons (four throws for a four
cylinder engine). Oil-ways are drilled through the shaft to transfer the lubricating oil onto the
bearing surfaces. Plain Bearings are used to enable the high reciprocating loads to be carried. |
The oil-ways can also be used to carry oil for the operation of a variable pitch Propeller. The crankshaft is accurately balanced to minimize vibration, however, when a shaft has to
transmit a torque or twisting moment it must flex to some extent and spring back again when
released. If the shaft must have a lot of kinks in it to provide the crank throws, the twisting
moments are hard to resist and perceptible deflection may take place. In the case of a radial engine, several cylinders may be connected to a single throw, and a
horizontally opposed engine may have only two pistons connected to one crank-pin. |
The repeated applications of force to which the crankshaft is subjected may set up oscillations
as the shaft recovers its original shape between power impulses. At certain speeds the impulses
may coincide with the natural vibration period of the shaft and give very rough running even
in an engine which is in good mechanical balance. For these reasons the shafts should be as
short as possible and adequately supported and counter-weighted to minimize these torsional
effects. In any event, many engines have rpm ranges which are prohibited for prolonged use
(Critical rpm) to prevent unnecessary vibration. |
This is indicated by a Red Arc on the rpm
indicator. Piston Engines - General
2
26
2
Piston Engines - General
It was previously stated that increasing the number of cylinders improves the power output
and makes the engine run smoother. This is because there are more power strokes in the 720°
of crankshaft rotation. This is called the Firing Interval. |
Four cylinders are generally regarded
as the minimum number to give reasonable firing interval. The firing interval for any engine
can be found by dividing 720° by the number of cylinders of the engine. i.e. 4 cylinder =180°
and a 6 cylinder engine = 120°. |
The crankshaft and cylinder arrangement will also determine the order in which the cylinders
fire. This is called the Firing Order of the engine. A typical four cylinder engine could have a firing order of 1-3-4-2. The cylinders do not fire
consecutively as this reduces the load and vibration on the crankshaft. |
Note: Lycoming firing order is 1-3-2-4. Connecting Rods
The connecting rods transmit the forces of combustion to the crankshaft; they convert the
linear movement of the pistons into rotary movement of the crankshaft. A connecting rod is
usually made of H section high tensile steel, to combine lightness with the strength necessary
to withstand the compressive and tensile loads imposed as the piston changes direction. The
rod is connected to the crank-pin of the crankshaft by a large circular bearing at the Big End
of the rod. |
The Pistons
Generally made of aluminium alloy, the piston forms a sliding plug in the cylinder and transmits
the force of the expanding gases via the connecting rod to the crankshaft. Bosses are formed
to house the Gudgeon Pin which fastens the piston to the Small End of the connecting rod. Circumferential grooves are machined in the piston to accommodate piston Rings which
provide the means of preventing pressure leakage past the piston in one direction and oil
leakage in the other. A number of piston rings can
be fitted to a piston. |
Their
arrangement will vary from
engine to engine, but will
be similar to the following
paragraphs, and Figure 2.13. The
Compression
Rings
prevent gas leakage into the
crankcase. They are fitted
into grooves cut into the
upper portion of the piston. Gas passing down between
the piston and the cylinder
wall forces a compression
ring down in its groove and
outwards against the cylinder
wall. |
A small amount of gas
will pass the top ring; so a second (and sometimes a third) compression ring is fitted. Figure 2.13 The piston and associated components
2
27
Piston Engines - General
2
Piston Engines - General
The Scraper Rings or Oil Control Rings prevent excess oil passing into the combustion chamber
and spread the oil evenly around the cylinder bore. They are designed so that the bearing face
is reduced in area and the bearing pressure consequently increased. The rings are generally made of a special grade of cast iron; the rings are sprung against the
cylinder walls. |
Cast iron has the ability to retain its elasticity when heated. It also has self-lubricating qualities
due to the graphitic content of the metal. This is desirable because during the power stroke
the walls of the cylinder are exposed to the hot combustion gases, and the thin film of oil is
burned away. Piston rings which are worn or stuck in their grooves will cause excessive blue smoke (burning
oil) to be ejected from the exhaust pipe. |
No dataset card yet